
Steven Lee/ Whitehead Institute
Discovering how to think about the brain and its associated diseases and disorders
This article is part of our series on neurobiology research at Whitehead Institute. Click here to view the entire collection.
In order to prevent and treat disorders and diseases of the brain, researchers first need to understand its underlying biology. This is not a simple task, because researchers cannot get a good look at cells inside a living brain. Additionally, many health issues that affect the brain are complex, with contributing factors that are not well understood.
Brain research at Whitehead Institute spans from brain development, to neurodegeneration, to regeneration, to the genetics of addiction. In addition to advancing our fundamental knowledge base, Whitehead Institute researchers are making discoveries and developing tools that they hope could lead to medical therapies. Read on to learn more about brain-related research at Whitehead Institute.
Uncovering disease risk and pathways
While some diseases and disorders can be attributable to a single genetic mutation or factor, many of those that affect the brain have a number of genetic and environmental factors potentially contributing. Every person affected may have a slightly different mix of contributing factors. This can make it hard for researchers to understand who is at risk, how symptoms are likely to progress, or what to target in order to effectively treat the symptoms. Whitehead Institute Member Olivia Corradin is developing strategies to better understand the often complex genetics underlying neurological and psychiatric disorders including multiple sclerosis and opioid use disorder.
Much of Corradin’s work focuses on genetic variants, DNA sequences that vary slightly from person to person. While many of these “spelling differences” in our genome have no consequence, some can increase a person’s risk of developing a particular disease. The focus of Corradin’s approach is to look at the networks surrounding genetic variants, rather than looking at the genetic variants on their own. Different DNA sequences regulate the activity of genes. By looking for patterns across networks of regulators that act on the same genetic variant of interest, Corradin has been able to identify which genetic variants are likely to be important to a disease and how they contribute, such as by determining in which cell types they act. These insights could lead to new strategies for treatment and prevention.

Oligodendrocytes, brain cells that Corradin found play a role in multiple sclerosis
Courtesy of Olivia Corradin/ Whitehead Institute
Corradin—along with lab technician Anna Barbeau and collaborator Daniel Factor, a senior scientist at Convelo Therapeutics—used such an approach to make discoveries about multiple sclerosis (MS), an autoimmune disease in which the body attacks the sheaths that wrap around and protect its own neurons, leading to potentially debilitating symptoms. They found that although immune cells play the most important role in MS, dysfunction in one type of brain cell is also implicated in the disease.
Corradin and colleagues from several research institutes, including Case Western Reserve University Professor Peter Scacheri, also looked at networks of gene regulators to learn more about opioid use disorder, which is linked to drug overdoses, a leading cause of accidental death in the United States. Researchers have struggled to identify the genes that most strongly increase risk of opioid use disorder, but with their approach, Corradin and colleagues identified five candidate genes that appear to be important contributors to opioid use disorder.
What makes brain function decline
Nucleotides are the basic building blocks of DNA and RNA. The five different types of nucleotide molecules are often abbreviated to their first letter; they are known as G, A, C, T, and U. When the same short sequence of nucleotides gets repeated too many times in a row—perhaps hundreds of times—this can cause a type of neurological disorder known as a repeat expansion disorder, a category that includes the neurodegenerative disorders amyotrophic lateral sclerosis (ALS) and Huntington’s disease.
Whitehead Institute Member Ankur Jain studies how excessive clumping of RNA molecules may contribute to repeat expansion disorders. Some parts of RNA are naturally prone to stick to each other. When an RNA molecule contains a long string of repeats, the RNA molecule tangles up, the same way that a long piece of tape will fold and stick to itself instead of lying straight. Affected RNA molecules may not only stick to themselves, but also tangle together to form large solid and gel-like clumps.
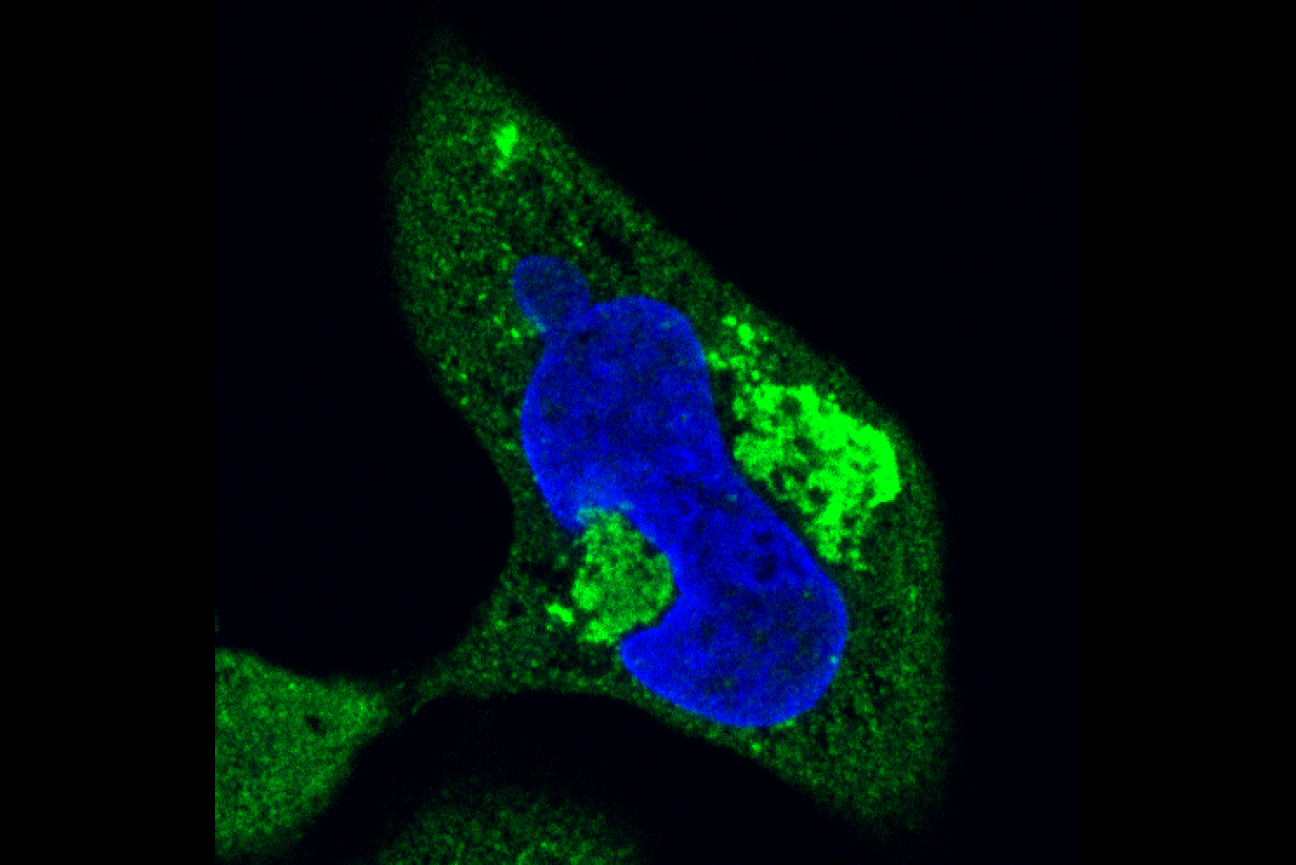
Hard clumps of repeat-containing RNA (bright green) and protein deform the nucleus (blue) of a cell.
Michael Das/ Whitehead Institute
Researchers have known that RNA clumps form in the nuclei of affected cells, but the clumps there appear to be relatively harmless. However, Jain lab researchers led by graduate student Michael Das found that the clumps can also occur in the cytoplasm, or main body of the cell. In this location, the clumps trap other molecules, preventing them from doing their jobs, and are toxic to the cell. The cytoplasmic clumps may be contributing to repeat expansion disorders, so Jain’s group is testing whether drugs that keep repeat-containing RNA from leaving the nucleus could be used as a therapy.
Jain’s group has also begun researching the role of small molecules in neurodegeneration. The group is particularly interested in polyamines, small positively-charged molecules that are prevalent in cells, but that decrease in number as a person ages. Loss of polyamines has recently been implicated in Parkinson’s disease, a progressive disorder of the central nervous system—but the exact connection is unclear. Pushkal Sharma, a graduate student in the Jain lab is trying to understand that connection.
Regenerating brain cells
When people lose nerve cells—the cells that send signals between the brain and the rest of the body—to illness or injury, that loss tends to be permanent. However, some animals are able to regenerate nerve cells. Understanding how these animals do this could provide useful insights into what prevents humans from doing so, and could provide clues to promoting healing or even regrowth of lost nerve cells.
Whitehead Institute Member Peter Reddien studies the regenerative planarian (Schmidtea mediterranea), a freshwater flatworm. Planarians can regrow their entire bodies, and Reddien lab researchers have uncovered many of the mechanisms and principles of planarian regeneration. After the researchers studied how the animal regrows its eyes, they were left with a new question: how does the planarian connect its new eyes to its brain?

Fluorescent image of the visual system of the planarian Schmidtea mediterranea. Visual axons and photoreceptor neurons shown in cyan, guidepost-like cells shown in magenta.
Lucila Scimone/Whitehead Institute
Axons are the wires between the eye and brain, and during development, or regeneration, they must accurately route their way through the body to connect the two. Most animals have “guidepost cells” that exist during their embryonic development to point axons in the right direction. However, these cells are typically not present in adulthood, when they are no longer needed. Reddien lab scientist Lucila Scimone and postdoc Kutay Deniz Atabay found that planarians maintain guidepost cells as adults, so that axons can always find the right route. The researchers also found that guidepost cells in planarians are muscle cells, adding to the lab’s list of important roles played by muscle in regeneration.
Recreating the brain
Since brain cells are not easy to study in a living brain, researchers are developing ways to more accurately recreate brain cells and brain-like environments in the lab. Whitehead Institute Founding Member Rudolf Jaenisch’s research group has developed several processes to model the brain. They begin by taking cells from people with a neurological disorder or disease of interest and introducing them to molecules that coax them into stem cells. They then use a cocktail of genetic signals to trigger the stem cells to turn into whatever brain cell type they are interested in studying. The Jaenisch lab has been the first to create several stem-cell derived cell types, including microglia, the brain’s immune cells.
Jaenisch lab researchers have used several strategies for improving the accuracy of stem-cell derived brain cell models, including coming up with new recipes to make their brain cells closer matches to the body’s cells, as well as growing cells in “organoids,” three-dimensional structures that can imitate some of the body’s complexity, such as interactions between cells, rather than simply studying cells in two dimensions in a dish.
The researchers have used their models to study microcephaly, or the development in a fetus of abnormally small head size, which is often accompanied by abnormal brain development and a range of related symptoms; and neuroblastomas, brain tumors that begin during fetal development.
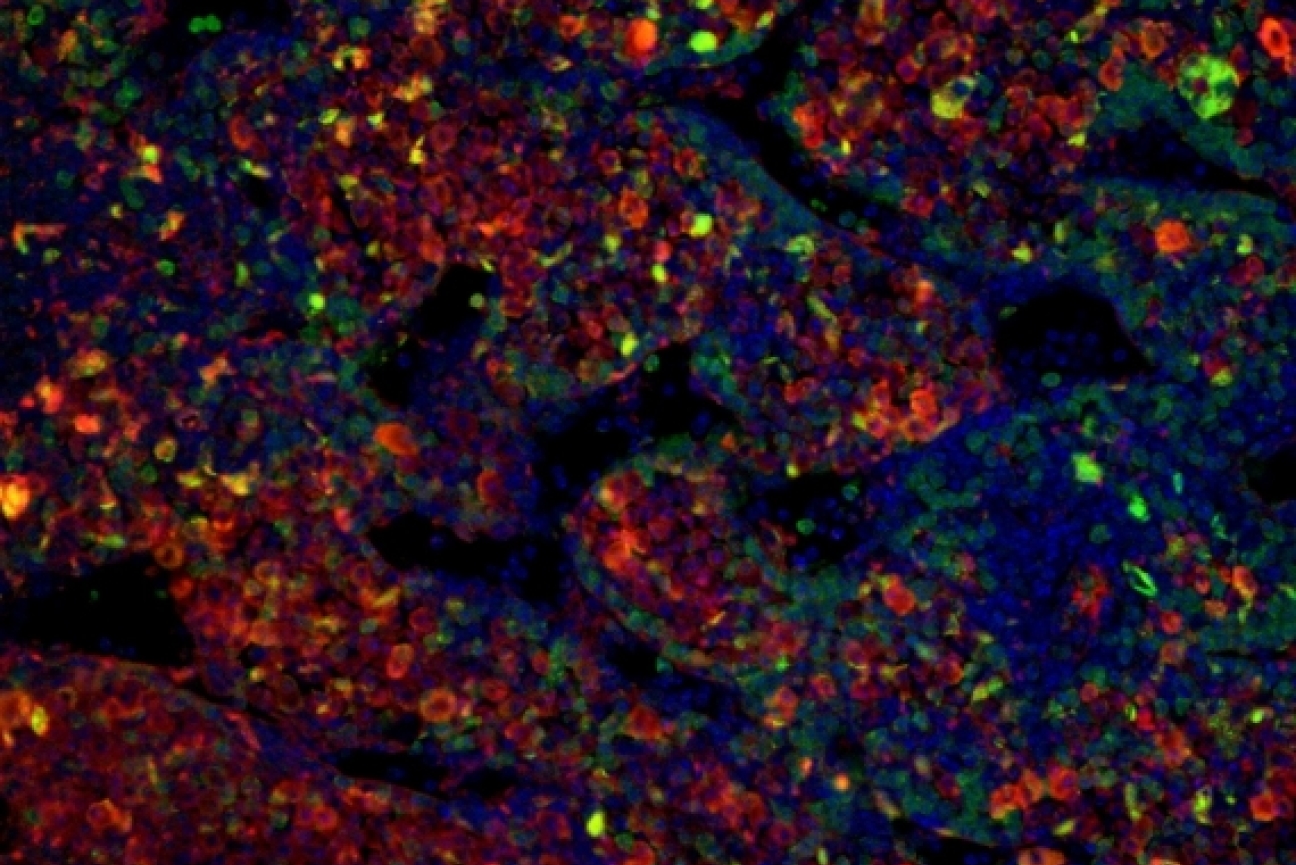
Malkiel Cohen/ Whitehead Institute
Jaenisch’s group also studies developmental disorders that cause intellectual disability, including fragile X syndrome and Rett syndrome, for which there is currently no cure. Each of these disorders is caused by mutations to a single gene. People who are affected have a copy of the disease gene in their cells that could function normally except that it has been deactivated. Former Jaenisch lab postdoc Shawn Liu and colleagues developed an approach that uses a modified version of CRISPR to turn genes off or on. Using this approach, Liu and colleagues were able to restore affected neurons to normal activity in cell models of fragile X and Rett syndrome. The researchers are further developing the tools in hopes that this approach could be used to treat people with these disorders.
Researchers in Jaenisch’s lab and colleague Whitehead Institute Member Richard Young’s lab are also tackling Rett syndrome from other angles. Researchers in the Young and Jaenisch labs, led by former Young lab graduate students Charles Li and Eliot Coffey, found that many of the mutations to the disease gene in Rett syndrome disrupt its protein product’s ability to form condensates, or droplets that corral and coordinate the actions of certain cellular machinery. Jaenisch lab researchers are following up on this work to see if targeting condensates could be a viable therapeutic strategy for Rett syndrome. Additionally, former Jaenisch lab postdoc Xing Tang helmed a project that identified promising drug candidates that could help treat Rett syndrome, and Jaenisch lab postdoc Danielle Tomasello is studying the role in Rett syndrome of astrocytes, a type of brain cell implicated in the disease.

Immunofluorescence microscopy of MeCP2 neurons used to learn about the role of condensates in Rett syndrome.
Charles Li/ Whitehead Institute
A deep dive into deep sleep
In periods of food scarcity or difficult environmental conditions, many mammals are able to enter a dormant state called torpor, which can be brief or last for a season, like hibernation. In this state, animals’ body temperatures drop and their metabolisms slow, reducing their need for energy or food. Whitehead Institute Member Siniša Hrvatin studies how animals enter and maintain these states, including how the brain regulates the initiation of torpor. Hrvatin wants to understand the mechanisms behind torpor to see whether it could be induced in humans in order for us to reap its benefits. Not only does torpor temporarily reduce energy requirements, but it may have long-term effects such as slowing disease progression, tissue damage, and the process of aging.
As a postdoctoral researcher at Harvard Medical School, Hrvatin identified the region of the brain that regulates torpor in mice. A group of neurons in the region of the brain called the hypothalamus, when stimulated, caused mice to undergo the same changes in metabolism and body temperature that they normally undergo during torpor. Identifying these neurons helps to illuminate how torpor is regulated, and also provides researchers with a way to trigger torpor in mice without having to put them in the starvation conditions that lead to torpor in the wild.
In his lab at Whitehead Institute, Hrvatin continues to study torpor in different animals, including further investigating how it is regulated in the brain. Listen to this podcast to hear Hrvatin discuss his current research, as well as his discoveries leading up to it.
Keeping the brain in mind
Although researching the basic biology of the brain poses many challenges, Whitehead Institute researchers continue to take innovative approaches to understand how the brain functions in health and disease, moving us closer to therapies that could treat or hopefully even one day prevent brain diseases and disorders.
Contact
Communications and Public Affairs
Phone: 617-452-4630
Email: newsroom@wi.mit.edu