Uncovering nature's riches
Probing the origin and evolution of plant metabolism
Jing-Ke Weng, a Member of Whitehead Institute and Assistant Professor of Biology at MIT, and his lab are focused on understanding how plants’ specialized metabolism evolved medicinal properties and how we can use new technologies to understand their origins.
Traditional Chinese medicine is at the heart of Chinese culture
The transition of plants onto land drove their metabolic evolution
Plants came onto land about 500 million years ago. This was a remarkable transition and many metabolic systems were established at the time to support this shift. These metabolic pathways were later expanded and adapted to better equip plants to deal with the challenges they encountered on land, such as self-defense.
As one means of self-defense against being eaten by land animals, some of the compounds produced by plants are toxic.
Other compounds plants produce help them manage ultraviolet radiation from the sun, or interact with insects that may pollinate them. Plants have to adapt metabolically to deal with quite a few challenges without being able to move around. This is the principle behind plant metabolic evolution and the origin of many of the medicinal compounds in plants.

Image courtesy of Wellcome Images.
Plants have to adapt metabolically to deal with quite a few challenges without being able to move around.
Ancient wisdom about healing plants
Multiomic approaches to understand medicinal plant chemistry
Golden root
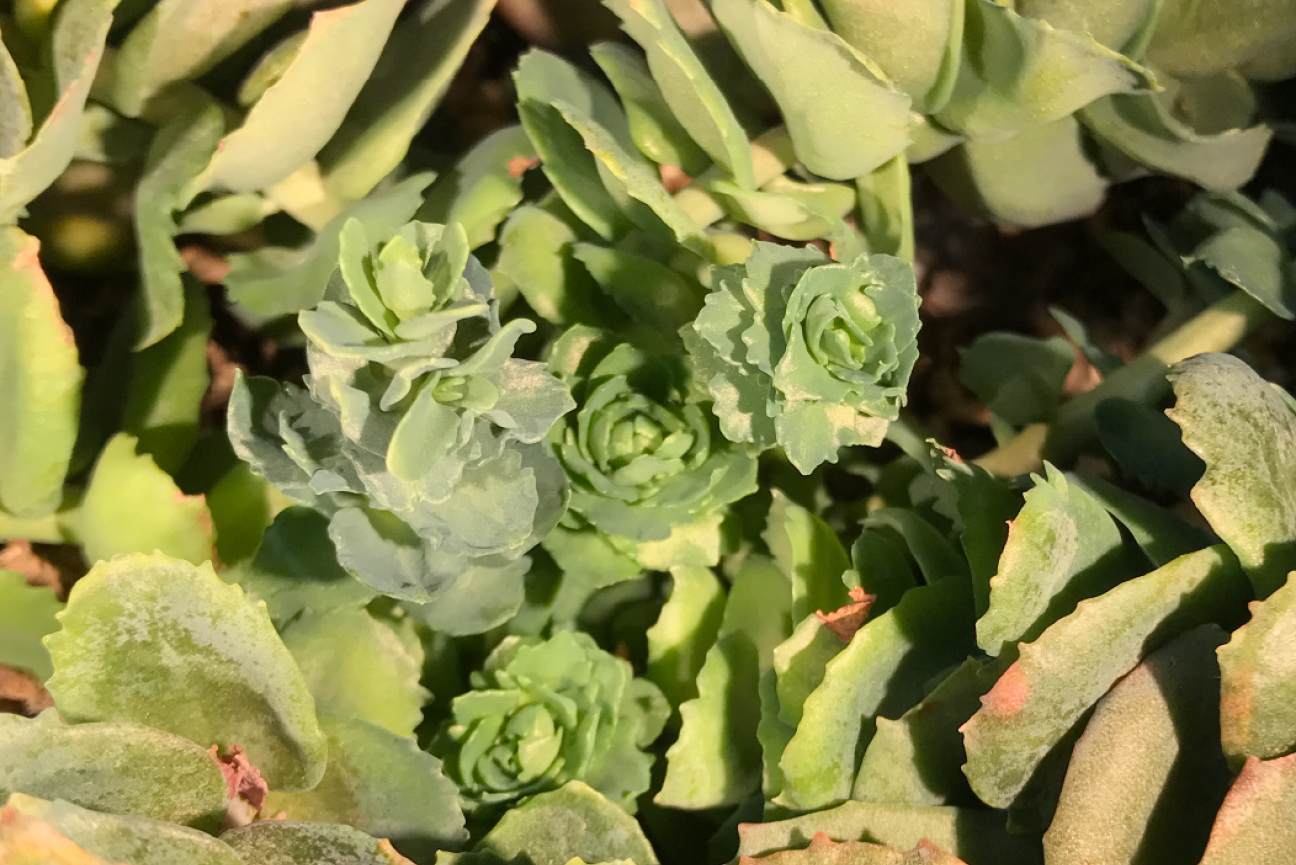
Golden root: Sustainable engineering approaches to spare an endangered plant
When Whitehead Institute Member Jing-Ke Weng’s mother fetched some backyard herbs to treat her sick son, she was one of the hundreds of millions of people globally each year who rely on plant-based medicine. Unlike the medicinal plants thriving in the Wengs’ yard, however, the vast majority of medicinal plants are harvested from the wild. With such high demand comes a concern of overharvesting, environmental impact, and even extinction. But Weng’s research may alleviate these environmental pressures by transplanting the production of medicinal compounds into unrelated host organisms, thereby removing herbal medicine’s reliance on the plant.
Golden root, also called Tibetan ginseng, typically grows in high-altitude, arctic environments, such as Tibet. It is well known in Eastern cultures as a panacea to increase endurance, boost immunity, counter the effects of high altitude, and alleviate depression. The plant produces a variety of chemical substances, particularly salidroside, which have garnered interest in the biomedical research community for their potential therapeutic effects.
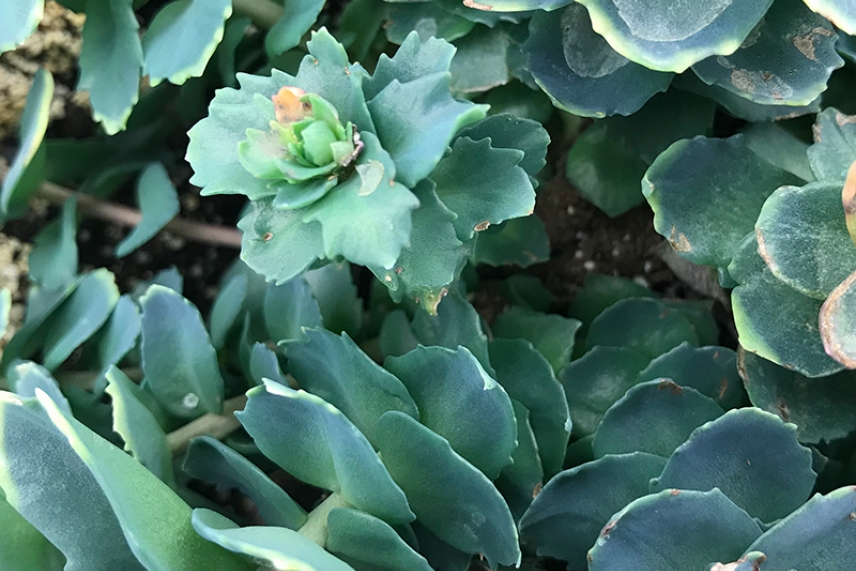
Golden root grows in harsh, arctic climates.
Image courtesy of Jing-Ke Weng.
“People have tried to farm golden root, but the medicinal value is much lower because the plants make much less salidroside when cultivated outside of their normal habitat,” says Weng.
That means collecting enough salidroside to fuel scientific studies is largely impossible, without risking the viability of these plants and their surroundings. So Weng and his team, including postdoc Michael Torrens-Spence, set out to find a better way. “If we can figure out how plants make these high-value natural products, then we can devise sustainable engineering approaches to recreate such molecules—we won’t have to destroy nature in order to harness its riches,” says Torrens-Spence.
Torrens-Spence and his colleagues used a systematic multiomics approach to characterize various tissues from a three-month-old, greenhouse-grown golden root plant. By correlating the active genes with the abundance of key metabolites between various tissue types, the researchers created a massive biochemical catalog of the plant’s tissues.
The researchers then mined these data and matched the likely biochemical precursors of salidroside with the candidate genes (and their corresponding enzymes) responsible for those compounds’ synthesis. This approach allowed Weng and his team to create a kind of draft blueprint of how salidroside is made in nature.
To test the validity of this draft blueprint—and the molecular players from the golden root plant that comprise it—the scientists turned to two well-studied laboratory organisms: baker’s yeast (Saccharomyces cerevisiae) and the tobacco plant (Nicotiana benthamiana). Normally, these organisms do not make salidroside. But if the researchers’ model was correct, by inserting the candidate genes involved in salidroside synthesis Weng and his colleagues should be able to bestow that special property upon them.
That is precisely what the researchers did. Using three key enzymes they identified through their “-omics” approach, including 4HPAAS (4-hydroxyphenylacetaldehyde synthase), 4HPAR (4-hydroxyphenylacetaldehyde reductase), and T8GT (tyrosol:UDP-glucose 8-O-glucosyltransferase), they engineered yeast and tobacco plants with the capacity to make salidroside. Notably, this biochemical pathway for synthesizing salidroside involves three enzymes, rather than four, as had previously been proposed.
“With this exciting method, we can systematically unlock the biochemistry that is the foundation of plant-based medicine,” says Weng. “That capability will accelerate biomedical studies of these unique compounds, define their potential therapeutic uses, and ultimately curtail the overharvesting of these precious and endangered plants.”
Additional information about this work can be found in the researchers’ recent Molecular Plant paper.
Goji berry

Lessons from the goji berry
The goji berry (Lycium barbarum) and gympie-gympie (Dendrocnide moroides) plants would seem to be a study in opposites. Marketed as a “superfruit”, goji products line health food stores’ shelves: dried goji berries, goji berry juice, goji berry snack bars, chocolate covered goji berries, and even goji berry anti-aging skin cream. In traditional Chinese medicine, various parts of the beneficial plant are used to treat hypertension, diabetes, tuberculosis, poor eyesight, and aging.
Gympie-gympie, which is also known as Australian stinging nettle or the suicide plant, is on the other end of the spectrum. Brushing against it causes the tiny hairs covering the plant to inject the powerful neurotoxin moroidin, which causes a rash and excruciating pain that can last for weeks or even years.
Yet the two plants are linked at the molecular level: goji produces lyciumins, benign peptides-- short amino acid sequences-- that are structurally similar to gympie-gympie’s neurotoxin. By applying natural products chemistry to lyciumins and moroidin, Roland Kersten hopes to identify novel, powerful painkillers.
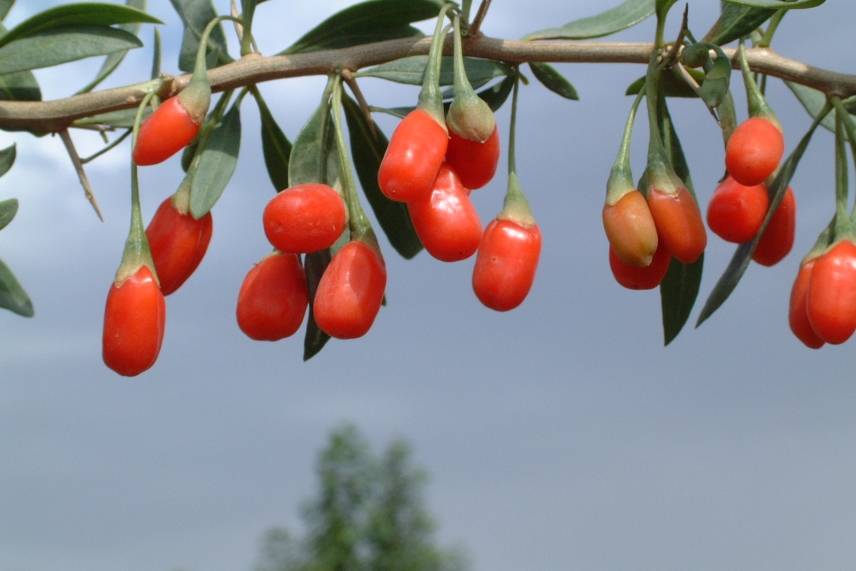
Goji berries ripening. The goji berry plant provides an attractive model to study plant peptide biochemistry.
In the past, biochemists have tweaked naturally occurring pain-causing molecules to block pain at the same receptor. For this reason, moroidin’s interactions with its pain receptor target are of particular interest to Kersten, a postdoctoral researcher in Whitehead Institute Member Jing-Ke Weng’s lab. Because the pain associated with moroidin persists for so long, the neurotoxin most likely binds very tightly to its target. And if the moroidin target is not an opioid receptor, then a moroidin-related analgesic might be non-addictive.
Kersten wanted to study moroidin and its potential as an analgesic template, but gympie-gympie is simply too dangerous and difficult to work with. Instead, he turned to the more innocuous goji for some aspects of his research. He identified the precursor gene for goji’s lyciumins and searched through genome databases to see what plants, if any, had similar genes. To Kersten’s amazement, 18% of the known plant genomes he checked had hits for a similar peptide precursor sequence, including potato, pepper, eggplant, amaranth, beets, and legumes. For Kersten, this work showcases the power of genome-based drug discovery for unearthing natural products chemistry in plants.
By analyzing and comparing how the goji and gympie-gympie compounds are produced, Kersten hopes to gain insight into the plants’ production processes. With a better understanding of how lyciumins and moroidin are synthesized, Kersten could then alter those
compounds’ structure indirectly by modifying their production or directly by varying their gene precursors. The result would be a library containing millions of possible molecules that could then be screened for analgesic traits or against pathogens—and the promise that something good can come from gympie-gympie.
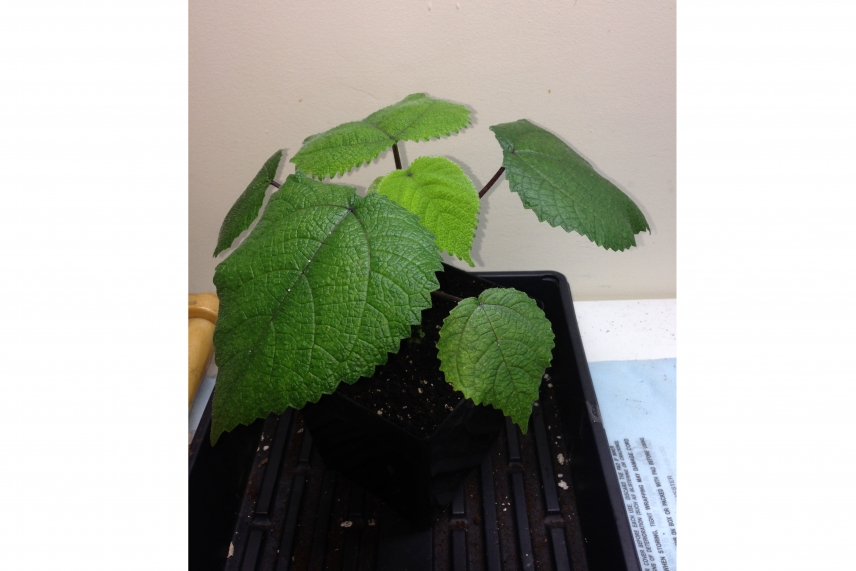
The gympie gympie plant may look innocuous, but its stinging nettles can cause days or weeks of pain.
Image courtesy of Roland Kersten.
Side note: Gympie-gympie has been difficult for Kersten to work with from the outset. Vendors do not sell the plant, so Kersten had to acquire seeds from a hobby botanist. Even housing a specimen is not easy. Because it is so noxious, gympie-gympie is not allowed in Whitehead’s greenhouse. Instead, a plant resides at Kersten’s home, covered by a plastic dome to prevent his cats—or anyone else—from accidentally grazing it. When the gympie-gympie plant needs to be repotted or requires other tending, Kersten protects himself with multiple layers of latex gloves. So far, neither he nor his cats have been injured.
Well-known drugs that are plant-derived
Kava
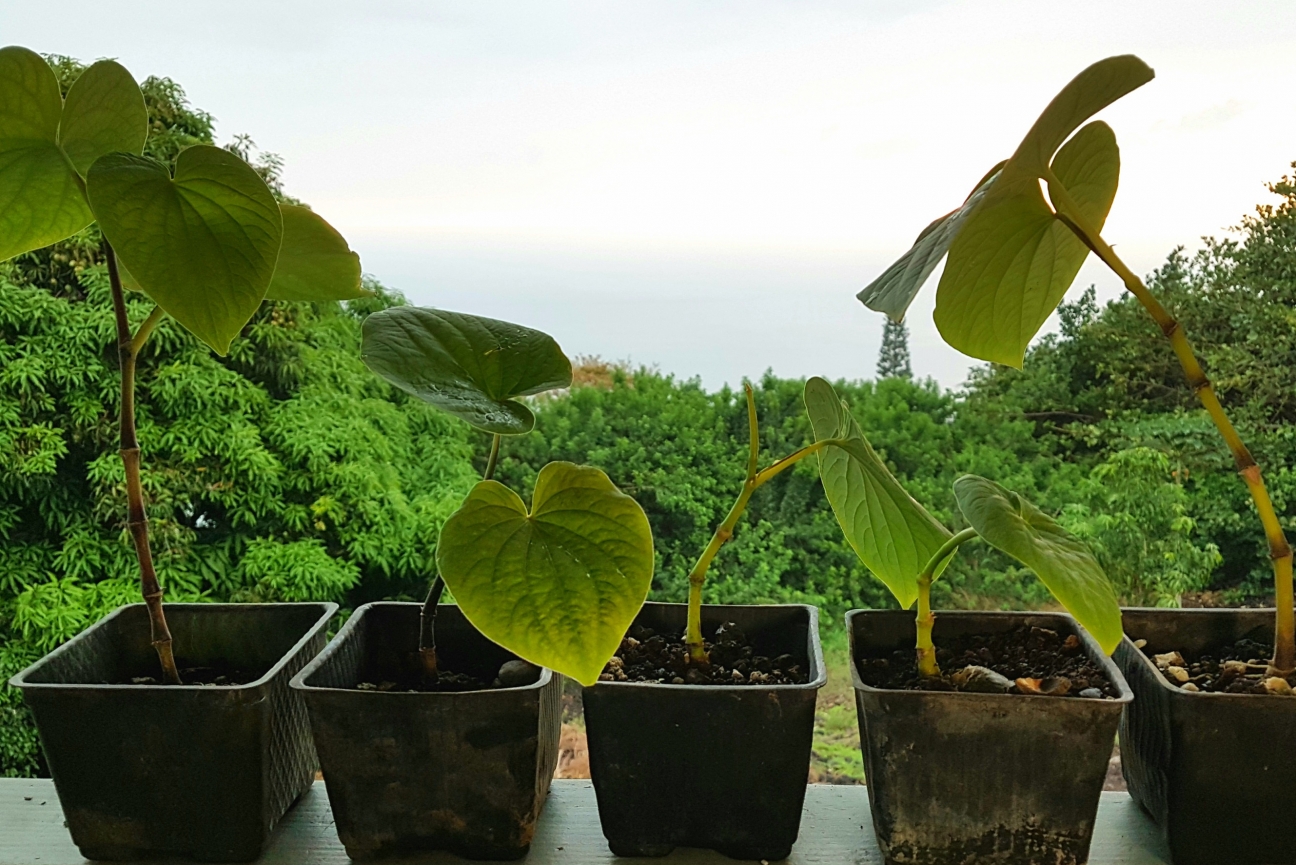
A cold brew prepared from the root of the Polynesian kava plant (Piper methysticum) has been consumed for its relaxing and calming effects during social rituals and ceremonies throughout the Pacific Islands for thousands of years.
The compounds in kava responsible for its anti-anxiety properties are called kavalactones. Kavalactones’ non-addictive effects are exerted through mechanisms distinct from those of opioids and certain antidepressants, making it attractive as a potential therapeutic or adjunct therapeutic for the millions of people suffering from anxiety disorders. Despite its promise, however, there are challenges in translating this ancient panacea into a modern pharmaceutical. Kava is a tropical plant that is difficult to cultivate outside of its native environment and further, it must be grown for five years in order for the roots to accumulate sufficient quantities of kavalactones for harvest.
Tomáš Pluskal, a postdoc in Jing-Ke Weng’s lab sought to circumvent these obstacles using a gene discovery-based approach. Tomas wanted to learn the complete biosynthetic pathways, starting with the genes encoding the enzymes, from which the compounds with psychoactive properties are synthesized. This allows him to then use this information to replicate their synthesis and produce the compounds at scale in a more tractable system, such as baker’s yeast or E. coli, an important step for producing sufficient material to study without having to grow and destroy kava plants and also a way to produce kavalactones at a pharmaceutically-relevant scale.
Moving kavalactone production from the plant into a “biofactory” such as yeast or bacteria also provides the opportunity to use metabolic engineering approaches to optimize the compounds for therapeutics.
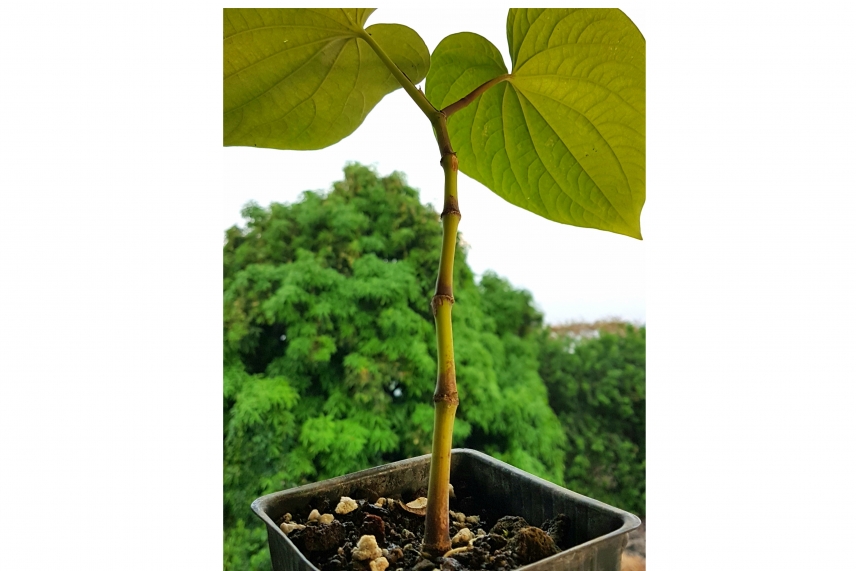
Kava is a tropical plant that is difficult to grow outside of its native environment.
Photo courtesy of Randy Travis.
Moving kavalactone production from the plant into a “biofactory” such as yeast or bacteria also provides the opportunity to use metabolic engineering approaches to optimize the compounds for therapeutics. Once the biosynthetic components are known, it is possible to mix and match within the context of a biofactory system for optimal properties, combining enzymes from the original plant with functionally appealing enzymes from another plant. This way, the chemical structures of kavalactones can be altered to increase their solubility or to increase their binding to target receptors, resulting in a longer effect.
Tomáš purchased the plant from a vendor in Hawaii and had it shipped to Boston. Unfortunately, the tropical plant did not survive for long. It did survive long enough, however, for him to collect plant tissue for sequencing and chemical analysis and eventually identify the exact genes that encode kavalactones responsible for the plant’s psychoactive properties. Tomáš was able to deduce, starting from the sequencing data, the kavalactone biosynthetic pathway consisting of seven enzymes and showed that it is feasible to engineer their production, as well as the production of variants, in yeast, bacteria, and a transformable model plants. This is a powerful example of how gene-based drug discovery can parlay knowledge gleaned from medicinal plants into potential new therapeutics.
Perspective
Combining ancient wisdom with modern approaches, scientists are ushering in a new era in plant-based therapeutics based on a better understanding of plants' specialized metabolism and its evolution.
Acknowledgements
We would like to acknowledge Jing-Ke Weng, Tomáš Pluskal, Michael Torrens-Spence, and the members of the Weng lab. Images included in healing plants video footage courtesy of Wiki Loves Earth 2016, José Luiz Bernardes Ribeiro (CC BY-SA 4.0) Wellcome Images, Ji Elle (CC SA 4.0).
Contact
Communications and Public Affairs
Phone: 617-452-4630
Email: newsroom@wi.mit.edu